Photodiodes
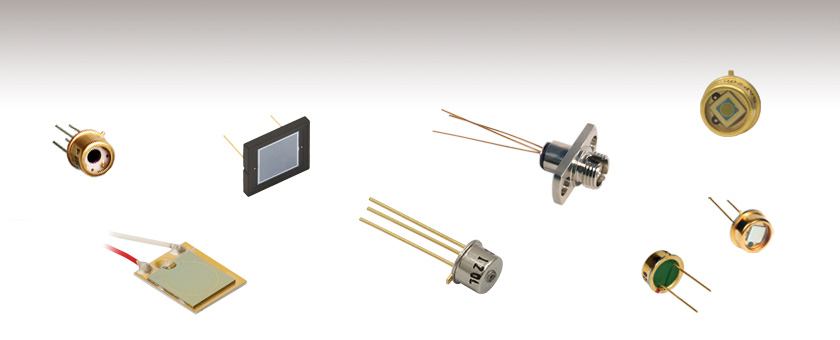
- Si, InGaAs, Ge, and Dual Band (Si/InGaAs) Detectors Available
- Available in TO Can, FC Connector, and Flat Wafer Body Styles
- Available in Hermetically Sealed Packages
DSD2
FDS10X10
FDG05
FGA01
FGA01FC
FDG03
FGA21
Front
Back

Please Wait
Mounted and Unmounted Detectors |
---|
Unmounted Photodiodes (200 - 2600 nm) |
Calibrated Photodiodes (350 - 1800 nm) |
Mounted Photodiodes (150 - 1800 nm) |
Thermopile Detectors (0.2 - 15 µm) |
Photovoltaic Detectors (2.0 - 10.6 µm) |
Pigtailed Photodiodes (320 - 1000 nm) |
Features
- Si, InGaAs, Ge, and Dual Band (Si/InGaAs) Unmounted Photodiodes Available
- Wavelength Ranges from 200 to 2600 nm
Thorlabs stocks a wide selection of photodiodes (PD) with various active area sizes and packages. Discrete PIN junction photodiodes include indium gallium arsenide (InGaAs) and silicon (Si) materials. Germanium (Ge) photodiodes, which are based on an N-on-P structure, are also available.
Our fastest photodiodes are the FDS015, FDS02, and FDS025 Si photodiodes. The FDS015 Si photodiode has a 35 ps rise time and a 0.65 pF junction capacitance, making it the highest speed, lowest capacitance photodiode offered below. Alternatively, the FD11A Si photodiode has a dark current of 2 pA, making it our photodiode with the lowest dark current. The FD10D and FD05D are InGaAs photodiodes with high responsivity from 900 to 2600 nm, allowing detection of wavelengths beyond the normal 1800 nm range of typical InGaAs photodiodes. The DSD2 is a dual-band photodiode, which incorporates two photodetectors sandwiched on top of each other (Si substrate on top of an InGaAs substrate), offering a combined wavelength range of 400 to 1700 nm.
To complement our photodiode product line, we offer mounted photodiodes and a range of compatible photodiode sockets. Please note that the PDs sold below are not calibrated, meaning responsivity will differ slightly from lot to lot; refer to the Response Variation tab for more information. We also offer calibrated photodiodes, which come with with NIST-traceable calibration, to correct for the differences in responsivity. Many of our photodiodes can be reverse voltage biased using the PBM42 DC Bias Module for faster speed and higher optical power detection.
For information on the photodiode saturation limit and the noise floor, as well as a collection of Thorlabs-conducted experiments regarding spatial uniformity (or varying responsivity) and dark current as a function of temperature, refer to the Lab Facts tab. This tab also outlines the theory and methods we use to define the specifications of our photodiodes. For example, the Noise Equivalent Power (NEP) as a Function of Temperature section provides background on NEP values specified by shot noise and thermal noise. With zero bias (Photovoltaic Mode), the NEP is specified by the thermal noise only, which is caused by the shunt resistance of the photodiode. The Photodiode Tutorial provides more general information regarding the operation, terminology, and theory of photodiodes.
Inhomogeneity on the edge of an active area of the detector can generate unwanted capacitance and resistance that distorts the time-domain response of a photodiode. Thorlabs therefore recommends that the incident light on the photodiode is well centered on the active area. This can be accomplished by placing a focusing lens or pinhole in front of the detector element.
Thorlabs offers spectral-flattening filters that are designed to improve the response uniformity of our silicon photodiodes. Click here to learn more.
The responsivity of a particular photodiode varies from lot to lot. Due to this, the photodiode you receive may have a slightly different response than what is represented below. For example, to the right, a graph for the FDS1010 photodiode shows the extent that the response may vary. This data was collected from 104 photodiodes. Minimum, Average, and Maximum responsivity was calculated at each data point and has been plotted.
To view typical responsivity vs. wavelength data for each individual photodiode, please click the buttons in the product specifications tables below.
Photodiode Tutorial
Theory of Operation
A junction photodiode is an intrinsic device that behaves similarly to an ordinary signal diode, but it generates a photocurrent when light is absorbed in the depleted region of the junction semiconductor. A photodiode is a fast, highly linear device that exhibits high quantum efficiency based upon the application and may be used in a variety of different applications.
It is necessary to be able to correctly determine the level of the output current to expect and the responsivity based upon the incident light. Depicted in Figure 1 is a junction photodiode model with basic discrete components to help visualize the main characteristics and gain a better understanding of the operation of Thorlabs' photodiodes.
Figure 1: Photodiode Model
Photodiode Terminology
Responsivity
The responsivity of a photodiode can be defined as a ratio of generated photocurrent (IPD) to the incident light power (P) at a given wavelength:
Modes of Operation (Photoconductive vs. Photovoltaic)
A photodiode can be operated in one of two modes: photoconductive (reverse bias) or photovoltaic (zero-bias). Mode selection depends upon the application's speed requirements and the amount of tolerable dark current (leakage current).
Photoconductive
In photoconductive mode, an external reverse bias is applied, which is the basis for our DET series detectors. The current measured through the circuit indicates illumination of the device; the measured output current is linearly proportional to the input optical power. Applying a reverse bias increases the width of the depletion junction producing an increased responsivity with a decrease in junction capacitance and produces a very linear response. Operating under these conditions does tend to produce a larger dark current, but this can be limited based upon the photodiode material. (Note: Our DET detectors are reverse biased and cannot be operated under a forward bias.)
Photovoltaic
In photovoltaic mode the photodiode is zero biased. The flow of current out of the device is restricted and a voltage builds up. This mode of operation exploits the photovoltaic effect, which is the basis for solar cells. The amount of dark current is kept at a minimum when operating in photovoltaic mode.
Dark Current
Dark current is leakage current that flows when a bias voltage is applied to a photodiode. When operating in a photoconductive mode, there tends to be a higher dark current that varies directly with temperature. Dark current approximately doubles for every 10 °C increase in temperature, and shunt resistance tends to double for every 6 °C rise. Of course, applying a higher bias will decrease the junction capacitance but will increase the amount of dark current present.
The dark current present is also affected by the photodiode material and the size of the active area. Silicon devices generally produce low dark current compared to germanium devices which have high dark currents. The table below lists several photodiode materials and their relative dark currents, speeds, sensitivity, and costs.
Material | Dark Current | Speed | Spectral Range | Cost |
---|---|---|---|---|
Silicon (Si) | Low | High Speed | Visible to NIR | Low |
Germanium (Ge) | High | Low Speed | NIR | Low |
Gallium Phosphide (GaP) | Low | High Speed | UV to Visible | Moderate |
Indium Gallium Arsenide (InGaAs) | Low | High Speed | NIR | Moderate |
Indium Arsenide Antimonide (InAsSb) | High | Low Speed | NIR to MIR | High |
Extended Range Indium Gallium Arsenide (InGaAs) | High | High Speed | NIR | High |
Mercury Cadmium Telluride (MCT, HgCdTe) | High | Low Speed | NIR to MIR | High |
Junction Capacitance
Junction capacitance (Cj) is an important property of a photodiode as this can have a profound impact on the photodiode's bandwidth and response. It should be noted that larger diode areas encompass a greater junction volume with increased charge capacity. In a reverse bias application, the depletion width of the junction is increased, thus effectively reducing the junction capacitance and increasing the response speed.
Bandwidth and Response
A load resistor will react with the photodetector junction capacitance to limit the bandwidth. For best frequency response, a 50 Ω terminator should be used in conjunction with a 50 Ω coaxial cable. The bandwidth (fBW) and the rise time response (tr) can be approximated using the junction capacitance (Cj) and the load resistance (RLOAD):
Noise Equivalent Power
The noise equivalent power (NEP) is the generated RMS signal voltage generated when the signal to noise ratio is equal to one. This is useful, as the NEP determines the ability of the detector to detect low level light. In general, the NEP increases with the active area of the detector and is given by the following equation:
Here, S/N is the Signal to Noise Ratio, Δf is the Noise Bandwidth, and Incident Energy has units of W/cm2. For more information on NEP, please see Thorlabs' Noise Equivalent Power White Paper.
Terminating Resistance
A load resistance is used to convert the generated photocurrent into a voltage (VOUT) for viewing on an oscilloscope:
Depending on the type of the photodiode, load resistance can affect the response speed. For maximum bandwidth, we recommend using a 50 Ω coaxial cable with a 50 Ω terminating resistor at the opposite end of the cable. This will minimize ringing by matching the cable with its characteristic impedance. If bandwidth is not important, you may increase the amount of voltage for a given light level by increasing RLOAD. In an unmatched termination, the length of the coaxial cable can have a profound impact on the response, so it is recommended to keep the cable as short as possible.
Shunt Resistance
Shunt resistance represents the resistance of the zero-biased photodiode junction. An ideal photodiode will have an infinite shunt resistance, but actual values may range from the order of ten Ω to thousands of MΩ and is dependent on the photodiode material. For example, and InGaAs detector has a shunt resistance on the order of 10 MΩ while a Ge detector is in the kΩ range. This can significantly impact the noise current on the photodiode. For most applications, however, the high resistance produces little effect and can be ignored.
Series Resistance
Series resistance is the resistance of the semiconductor material, and this low resistance can generally be ignored. The series resistance arises from the contacts and the wire bonds of the photodiode and is used to mainly determine the linearity of the photodiode under zero bias conditions.
Common Operating Circuits
Figure 2: Reverse-Biased Circuit (DET Series Detectors)
The DET series detectors are modeled with the circuit depicted above. The detector is reverse biased to produce a linear response to the applied input light. The amount of photocurrent generated is based upon the incident light and wavelength and can be viewed on an oscilloscope by attaching a load resistance on the output. The function of the RC filter is to filter any high-frequency noise from the input supply that may contribute to a noisy output.
Figure 3: Amplified Detector Circuit
One can also use a photodetector with an amplifier for the purpose of achieving high gain. The user can choose whether to operate in Photovoltaic of Photoconductive modes. There are a few benefits of choosing this active circuit:
- Photovoltaic mode: The circuit is held at zero volts across the photodiode, since point A is held at the same potential as point B by the operational amplifier. This eliminates the possibility of dark current.
- Photoconductive mode: The photodiode is reversed biased, thus improving the bandwidth while lowering the junction capacitance. The gain of the detector is dependent on the feedback element (Rf). The bandwidth of the detector can be calculated using the following:
where GBP is the amplifier gain bandwidth product and CD is the sum of the junction capacitance and amplifier capacitance.
Effects of Chopping Frequency
The photoconductor signal will remain constant up to the time constant response limit. Many detectors, including PbS, PbSe, HgCdTe (MCT), and InAsSb, have a typical 1/f noise spectrum (i.e., the noise decreases as chopping frequency increases), which has a profound impact on the time constant at lower frequencies.
The detector will exhibit lower responsivity at lower chopping frequencies. Frequency response and detectivity are maximized for
Summary
This tab contains a collection of experiments performed at Thorlabs regarding the performance of photodiodes we offer. Each section is its own independent experiment, which can be viewed by clicking in the appropriate box below. Photodiode Saturation Limit and Noise Floor explores how different conditions, including temperature, resistivity, reverse-bias voltage, responsivity, and system bandwidth, can affect noise in a photodiode's output. Photodiode Spatial Uniformity explores variations in the responsivity as a small-diameter light beam is scanned across the active area of the photodiode. Photodiodes with different material compositions are tested, and eight units of one silicon-based model are tested to investigate unit-to-unit variations. Dark Current as a Function of Temperature and Noise Equivalent Power (NEP) as a Function of Temperature describe how dark current and NEP, respectively, vary with temperature and how measurements are affected. Beam Size and Photodiode Saturation shows how the photodiode saturation point changes with the incident beam size and investigates several models to explain the results. Bias Voltage examines the effects of incident power on the effective reverse bias voltage of a photodiode circuit and verifies a reliable model for predicting those changes.
Photodiode Saturation Limit and Noise Floor

Click to Enlarge
Figure 1. Overview of the Photodiode's Response Curve,
Highlighting the Saturation Limit and the Noise Floor
We present laboratory measurements of the saturation limit and noise floor of a Thorlabs silicon photodiode. While all photodiodes function similarly, there are a number of parameters that affect the noise floor and saturation limit of a photodiode including the sensor temperature, resistivity, reverse bias voltage, responsivity, and system bandwidth. Here we investigated the effect of reverse bias voltage and load resistance within a silicon-based photodiode detection system. Increasing the reverse bias increased the saturation limit and had minimal effect on the noise floor. Decreasing the load resistance decreased the noise floor until reaching the noise of the measurement system, but also decreased the saturation limit. These results demonstrate some of the considerations necessary for choosing the reverse bias voltage and load resistance, and emphasize that noise sources within all of the components must be considered when creating a detection system.
For our experiment we used the FDS100 Si Photodiode as the photodiode under investigation. The collimated output of a fiber-pigtailed laser diode was used as the light source with output power from 0 to 50 mW. The collimated beam was incident upon a beamsplitter that transmitted the majority of the light to the photodiode under investigation and reflected the rest towards a reference power sensor. The photodiode response was then evaluated under various resistive loads and with different reverse bias voltages.
The plots to the right and below summarize the measured results for the various tested configurations. From these graphs the changes to the photodiode's linear response, noise floor, and saturation limit can be observed under different reverse voltage biases and load resistances. Figure 1 provides an overview of the photodiode response with a reverse voltage bias of 5 V and resistive load of 10 kΩ. The photodiode saturated at the upper limit of the response when the output photovoltage approached the reverse bias voltage. The noise floor at the lower limit of the response was a result of dark current and the thermal noise from the resistive load (Johnson noise). Figure 2 summarizes the results obtained using the photodiode with a 1 kΩ resistive load and various reverse bias voltages. It illustrates that the saturation limit can be raised by increasing the reverse bias voltage (within specification). Figure 3 summarizes the results from using the photodiode with a 5 V reverse bias voltage and various resistive loads. It illustrates that the slope of the photovoltage response increased as the load resistance was increased. Figure 4 summarizes the noise floor results obtained using a 0 V reverse bias voltage and various resistive loads. The noise floor increased when larger load resistances were used. It is important to note that the 1 kΩ data was measured above the theoretical Johnson noise due to the voltage noise within the measurement system. Minimal change in the overall noise floor was seen when using a 5 V reverse bias voltage. For details on the experimental setup employed and these summarized results, please click here.

Click to Enlarge
Figure 2. Photovoltage Dependence on Reverse Bias Voltage

Click to Enlarge
Figure 4. Noise Floor with Various Resistive Loads

Click to Enlarge
Figure 3. Response with Various Resistive Loads
Photodiode Spatial Uniformity

Figure 1: Responsivity, which describes the relationship between the strength of the photocurrent output by the photodetector and the optical power in the incident beam, is dependent on the wavelength of the incident light.
Do all regions of a photodiode's active area have the same responsivity?
The output signal strength from a photodetector is significantly affected by the optical power and wavelength of the incident light. A measure of this relationship is responsivity, which is the magnitude of the output photocurrent divided by the optical power of the incident light. While responsivity has units of ampere per watt, it also varies with wavelength. Often overlooked is that responsivity can also vary as different regions of the photodiode's active area are illuminated.
This work investigated the change in photodetector response as a light beam, with a diameter that was small in comparison to the active area of the photodiode, was scanned across the active area. A selection photodetectors, based on mounted gallium phosphide (GaP), silicon (Si), indium gallium arsenide (InGaAs), or germanium (Ge) photodiodes, were tested.
Responsivity is Wavelength Dependent1,2
One reason responsivity varies with respect to wavelength is the relationship among optical power, wavelength, and photo-generated electrons. A light beam illuminating a surface provides an optical power equal to the energy in the beam divided by the time the surface was illuminated. The energy in a single-wavelength beam equals the number of incident photons times the energy of a single photon. Decreasing the wavelength of the light increases the energy of the photons. If the wavelength is decreased, but the optical power is kept the same, there are fewer incident photons.
A single photon can generate no more than a single electron of photocurrent in these photodetectors. Due to this, reducing the wavelength of the incident light while holding its optical power constant results in a reduced photocurrent. Since responsivity relates the output photocurrent to the incident optical power, instead of to the number of incident photons per unit time, responsivity is lower at lower wavelengths. The responsivity curves plotted in Figure 1 are examples showing responsivity increasing with wavelength.
The upper wavelength of a photodetector's responsivity is limited by inherent properties of the semiconductor material. In order for an incident photon to contribute to the photocurrent, the photon must have enough energy to free an electron from its bonds to its host atom. Photons with wavelengths above the upper wavelength limit do not have enough energy. The semiconductor material composing the photodiode determines the wavelength of this limit.
Response May Vary as Different Regions of the Photodiode's Active Area are Illuminated1,2
An electron that has escaped the bonds of its host atom by absorbing the energy of a photon is a photo-generated electron. A photo-generated electron contributes to the photocurrent only if the electron is able to successfully travel from where it was generated, across the semiconductor material, and to an electrical contact. A photo-generated electron does not contribute to the photocurrent if it is re-absorbed by the semiconductor material before reaching a contact, which is a more likely outcome if the electron encounters a defect.
Defects are places where the semiconductor's crystal lattice is not perfect. These locations include dislocations, impurities, and voids in the crystal lattice. The external surfaces of a real crystal with finite dimensions are also imperfections, since a perfect crystal is infinite in length, height, and width. Defects in the semiconductor crystal absorb photo-generated electrons at a high rate and turn their kinetic energy into heat, rather than allow the electrons to contribute to the photocurrent.
Electrons generated near defects are more likely to be re-absorbed and less likely to contribute to the photocurrent. While the density of defects in a semiconductor can be minimized, the semiconductor crystals used in photodiodes are not perfect. It is also not unusual for the density of defects to vary throughout the volume of the semiconductor crystal, as well as for the growth of some semiconductor materials to be more prone to forming defects than others.
If the density of defects varies throughout the volume of the semiconductor material, the magnitude of the photocurrent generated within each region would be expected to be different. Since the photodetector's responsivity depends on the magnitude of photocurrent, a varying density of defects would result in the responsivity changing as different locations on the photodetector are illuminated. This effect was investigated using the procedure described in the following section.

Click to Enlarge
Figure 2: The setup used to measure the change in response across the active area of each photodiode included: a reflective collimator (A), an achromatic doublet lens (B) , vertically-mounted linear translation stage (C), the photodetector under test (D), and two horizontally-mounted translation stages (E) of which one is visible.
Experimental Setup and Results
Measurement Objectives
Several photodetectors were tested to investigate the uniformity of the responsivity across their active areas. The spatially dependent responsivities of one GaP-based, four Si-based, two InGaAs-based, and two Ge-based photodetectors were measured at selected wavelengths. Testing was conducted to investigate the spatial uniformity of the responsivity of:
- All nine photodetector models at a wavelength close to the wavelength of peak responsivity. (Results contained in the first expandable table below.)
- A Si-based, an InGaAs-based, and a Ge-based photodetector at three different wavelengths. (Results contained in the second expandable table below.)
- Eight different units of a single Si-based photodetector model at a wavelength close to the wavelength of peak responsivity. (Results contained in the third expandable table below.)
Experimental Setup (Components Labeled in Figure 2 are Labeled in the Following)
The experimental setup consisted of a light source (not shown) that was fiber-coupled to an RC12APC-P01 collimator (A), which was installed in a KM100T kinematic mount. An AC254-150-A achromatic doublet lens with a 150 mm focal length (B), which was installed in a ST1XY-D mount, focused the light on the active area of the photodetector (D), which was mounted with the help of a KB1P magnetic quick-release carriage set. Other components in the vertical arm included an LTS150 motorized linear translation stage (C), an XT66P1 vertical mounting plate, an XT66-500 66 mm construction rail, and four XT66C4 clamping platforms. The components in the horizontal arm included two previous-generation LNR50S motorized linear translation stages (E), an LNR50P3 XY adapter plate, a baseplate, and two BSC201 closed-loop stepper motor controllers (not shown), which scanned the beam across the active area of the photodetector (D). Most light sources were products from our Superluminescent Diode series. The exception was the 405 nm light source, which was an S3FC405 benchtop laser source.
Procedure
The diameter of the beam was scaled according to the active area of the photodetector, so that approximately 3600 measurements were taken in a 60 x 60 grid across the surface of each photodiode. The beam diameter used for each photodiode was between 50 µm and 500 µm, and the size used to make a particular measurement is included in the notes that accompany each plot in the following expandable tables. To view this information, click on the plot of interest.
During the scan, the position of the beam remained stationary, and the two LNR50S motorized stages were used to translate the photodetector in the two directions perpendicular to the optic axis. To ensure that the entire active area of the photodetector was tested, the dimensions of the scan area exceeded the dimensions of the active area.
No reverse bias voltage was applied to any of the photodiodes during this investigation.
Results (Click More [+] to Expand the Following Tables, and Click Less [-] to Hide Them)
The measurement data were normalized and then plotted with respect to the measurement position. The data in each measurement set were normalized with respect to the value at the center of the active area, which resulted in plots that show the variation of the responsivity across each photodetector's active area and allow the results from these nine different photodetector models to be compared. The plotted data were also processed to remove data points with values indistinguishable from noise. This was done to eliminate data points corresponding to measurements made outside of the active area of each photodetector. However, this approach also removed some measurements made within, but close to, the outer edges of the active areas. The lower photocurrent generated by these regions may have been due to the beam spot partially illuminating regions outside of the active area, as well as photocurrent suppressed by a higher density of defects near the edges.
The plots can be revealed by expanding the following tables. Each plot includes a dashed outline surrounding the central 90% of the active area of the photodiode. Under general use, the light beam should remain within this central 90% region to avoid edge effects. Click on each plot to reveal information about the active area of the photodiode and the size of the beam scanned across the active area.
Since these photodetectors represent a small sample size, these measurements are representative of the other photodetectors of its model, but results obtained using other units of the same model may vary. The measurements acquired for these photodetector units were repeatable, but no two semiconductor crystals are identical and unit-to-unit variations should be expected. The following tables show that:
- First Table: The variations of the responsivities across the active areas of the Ge-based and GaP-based photodetectors are greater than the variations of the responsivities across the active areas of the Si-based and InGaAs-based photodetectors. This suggests the defect densities of the Si-based and InGaAs-based semiconductor materials are more uniform than the defect densities of the Ge-based and GaP-based semiconductor materials.
- Second Table: The variations of the responsivities across the active areas of a Si-based, an InGaAs-based, and a Ge-based photodetector change with wavelength. For each photodetector, the minimum spatial variations of the responsivity occurred when the wavelength of light was near the wavelength of the peak value of responsivity.
- Third Table: Eight units of a single Si-based photodetector model had similar variations of spatial uniformity across their active areas.
1) All Nine Photodetectors: Uniformity of Response to Light with Near Peak Responsivity Wavelength |
---|
Photodetector Measured | Plot of Response Across the Sensor Area to Light of the Indicated Wavelength | Typical Responsivity Plots Measurement Wavelength Indicated |
|
GaP-Based Photodetector SM05PD7A: Mounted Photodiode FGAP71 |
![]() Click for Details |
![]() Click to Enlarge |
|
Si-Based Photodetectors SM05PD2A: Mounted Photodiode FDS010 and SM1PD2A |
![]() Click for Details |
![]() Click for Details |
![]() |
Si-Based Photodetectors SM05PD1A: Mounted Photodiode FDS100 and SM1PD1A: Mounted Photodiode FDS1010 |
![]() Click for Details |
![]() Click for Details |
![]() |
InGaAs-Based Photodetectors SM05PD5A: Mounted Photodiode FGA21 and SM05PD4A: Mounted Photodiode FGA10 |
![]() Click for Details |
![]() Click for Details |
![]() |
Ge-Based Photodetectors SM05PD6A: Mounted Photodiode FDG1010 and SM1PD5A: Mounted Photodiode FDG03 |
![]() Click for Details |
![]() Click for Details |
![]() |
2) Selected Si-, InGaAs-, and Ge-Based Photodetectors: Uniformity of Response of Each to Three Different Wavelengths of Light |
---|
Photodetector | Plots of Response Across the Sensor Area to Light of the Indicated Wavelengths | Typical Responsivity Plots Measurement Wavelengths Indicated |
||
Si-Based Photodetector SM1PD1A: Mounted Photodiode FDS1010 |
![]() |
![]() |
![]() |
![]() |
InGaAs-Based Photodetector SM05PD5A: Mounted Photodiode FGA21 |
![]() |
![]() |
![]() |
![]() |
Ge-Based Photodetector SM1PD5A: Mounted Photodiode FDG1010 |
![]() |
![]() |
![]() |
![]() |
3) Eight Si-Based SM1PD2A Photodetectors: Uniformity of Response to Light Near Peak Responsivity Wavelength Compared |
---|
Eight different Si-based SM1PD2A photodetectors were measured to compare the unit-to-unit variation of the response across the active area. Measurements were performed using 830 nm light, which is close to the wavelength of peak responsivity, as is indicted on the plot on the left. |
![]() Click to Enlarge |
||
![]() Click for Details |
![]() Click for Details |
![]() Click for Details |
![]() Click for Details |
![]() Click for Details |
![]() Click for Details |
![]() Click for Details |
![]() Click for Details |
References
[1] G. P. Agrawal, Fiber-Optic Communication Systems, 2nd ed., John Wiley & Sons, Inc., New York, 1997. (Particularly Chapter 4)
[2] A. Rogalski, K. Adamiec, and J. Rutkowski, Narrow-Gap Semiconductor Photodiodes, SPIE Press, Bellingham, WA, 2000.
Dark Current as a Function of Temperature
Measurements of dark current as a function of temperature were acquired for several unmounted photodetectors. As is described in the following section, dark current is a relatively small electrical current that flows in p-n junction photodetectors when no light is incident on the detector. Measurements were taken for silicon (Si), germanium (Ge), gallium phosphide (GaP), and indium gallium arsenide (InGaAs) reverse-biased photodiodes over temperatures from 25 °C to approximately 55 °C.

Figure 1: Current-Voltage Characteristic of a P-N Junction
Current-Voltage Characteristics of p-n Junction Photodiodes
The characteristic current-voltage relationship of p-n junction photodiodes, as diagrammed in Figure 1, possesses forward-biased and reverse-biased voltage regimes. In the reverse-biased voltage regime, in which p-n junction photodiodes are operated, a potential difference applied across the diode resists the flow of current. Ideally, if no light is incident on a reverse-biased photodiode, no current flows.
Under real-world conditions, random processes in the semiconductor material of the photodiode always generates current carriers (electrons and holes) that produce current. These current generation processes are not driven by the photogeneration of electrons and holes. Instead, they are largely driven by the thermal energy contained in the semiconductor material.[1] This dark current is generally small, but it is present when the photodiode is reverse biased and not illuminated. Dark current magnitudes vary for photodiodes of different material compositions; the efficiencies of the thermal generation processes depend on the type and crystal quality of the semiconductor used in the detector's sensing head. The magnitude of the dark current can be expected to increase as the temperature of the photodiode increases.
When a photodiode is illuminated, the current generated by the incident light adds to the dark current. The carriers in the photocurrent are generated by the energy contained in the photons of the incident light. Above a certain illumination threshold intensity, the magnitude of the photocurrent exceeds the magnitude of the dark current. When the photocurrent is larger than the dark current, the magnitude of the photocurrent can be calculated by measuring the total current and then subtracting the contribution of the dark current. When the photocurrent is smaller than the dark current, the photocurrent is undetectable. Because of this, it is desirable to minimize the levels of dark current in photodiodes.
For convenience, both dark current and photocurrent are discussed as being independent of voltage over a range of voltages when the photodiode is reverse biased; however, the current flowing in real-world reverse-biased photodetectors is not completely independent of voltage over any voltage range. Regardless of whether the diode is illuminated, the current will increase as the magnitude of the reverse-bias voltage increases. In addition, if the reverse-bias voltage is increased beyond a certain threshold, the photodiode will suffer reverse breakdown, in which the magnitude of the current increases exponentially and permanent damage to the diode is likely.
Experiment: Dark Currents Measured for Packaged Photodiodes
Dark currents were measured over temperatures of 25 °C to approximately 55 °C for four representative unpackaged photodiodes: the Si-based FDS1010, the Ge-based FDG50, the GaP-based FGAP71 (previous generation), and the InGaAs-based FGA10.

Click to Enlarge
Figure 2: The Nested Metal Box Test Fixture with Covers Removed and FGA10 Installed
Black insulating foam lines the interiors of both boxes.
A: Thermistor; B: FGA10; C: BNC-to-BNC Feedthrough; D: Outer Box; E: Inner Box; F: BNC-to-Triax Feedthrough; G: BNC-to-BNC Feedthrough

Click to Enlarge
Figure 4: An electrical circuit that included a resistive foil heater, TED8040 temperature controller, and two rectifying diodes controlled current flow through the resistive current heater as described in the text.

Click to Enlarge
Figure 3: The Test Setup
The custom-built temperature chamber is on the top of the cart, and the temperature control instrumentation that includes the PRO8000 and TED8040 cards is on the cart's lower shelf.
Experimental Setup
The experimental setup was designed to ensure a constant reverse-bias voltage across the photodiode, control the temperature of the detector, block light from reaching the detector, and establish an electrical path between the detector and the Keithley 6487 ammeter that isolated the measured current from extraneous electromagnetic interference (EMI) noise sources. Dark currents for these detectors can be on the order of nA, which made it important to control these conditions to ensure accurate measurements.
The detector enclosure consisted of a pair of nested aluminum metal boxes, which are shown with their covers off in Figure 2. Only one box would be required to create a light-tight environment for the photodiode, but an outer box was included in the setup to shield the inner box from EMI. The detector was placed inside the inner box, and it was electrically connected to the Keithley 6487 ammeter. The ammeter provided the required 5 V reverse-bias and received the output current signal. The electrical signals between the ammeter and the photodiode were routed through the two boxes using a combination of cables and feedthroughs. The 5 V bias voltage was connected to the photodiode using coax cables and BNC-to-BNC feedthroughs in the walls of both metal boxes. The current signal from the photodiode was connected to the BNC end of a BNC-to-Triax feedthrough integrated into the wall of the inner box. The signal was then routed through the outer box and to the Keithley 6487 using triax cables and a Triax-to-Triax feedthrough in the outer box. Triax cables were used because coax cables are poorly shielded, and using a coax cable to route the signal to the ammeter could introduce noise from EMI sources to the signal read from the detector. As the outer box shields the inner box from EMI, the noise introduced on the signal as it travels from the detector to the BNC-to-Triax feedthrough is mitigated.
Thermistors were used to monitor the temperatures of each photodiode continuously during the experiment. A piece of thermal tape was used to hold the thermistor flush against the TO cans of the FDG50, FGAP71, and the FGA10 during testing. The photosensor of the FDS1010 is mounted on a ceramic substrate, and when it was tested the thermistor was taped to the back of the substrate. The electrical connection between the thermistor and TSP01 temperature logger was performed using BNC cables, BNC-to-BNC bulkhead feedthroughs to route the signal out of the nested boxes, and a custom BNC-to-phono jack cable to connect to the temperature logger.
A custom temperature chamber based on a XE25C9 standard enclosure was used to conduct these tests. It is shown on top of the cart in Figure 3. The enclosure had a floor and four walls, and insulation was attached to all inner and outer surfaces. A lid for the enclosure was fashioned from a sheet of hardboard bordered with XE25 rails, and insulation was attached to the inner surface of the hardboard. Six resistive foil heaters were affixed to the walls of the XE25C9 enclosure, and they were driven using the PRO8000 fitted with six TED8040 thermoelectric cooler (TEC) controller cards placed on the bottom shelf of the cart in Figure 3. Each TED8040 was interfaced with a heater and a thermistor installed in the chamber. The reading from the thermistor determined the current sent to drive the heater. The chamber was not actively cooled; cooling was instead performed by withdrawing the current driving the heaters and, as an option, opening the lid of the chamber.
The TED8040 units are designed to be interfaced with TECs, which produce heat when current flows in one direction, and which provide cooling when the current flows in the opposite direction. Because of this, when the enclosure exceeded the setpoint temperature, the TED8040 units in this experimental setup did not cut off the driving current. They instead reversed the current flow in an effort to produce cooling. In contrast to TECs, resistive foil heaters generate heat regardless of the direction of current flowing through them. In order to divert the driving current from the heaters, an electrical circuit, diagrammed in Figure 4, that included two rectifying diodes was designed and implemented. Rectifying diodes allow current to flow only in one direction. When the temperature reading from the thermistor was lower than the setpoint temperature, current flowed in the direction of the red arrows and through the resistive heater, and heat was generated. When the temperature of the enclosure exceeded the setpoint temperature, the TED8040 controllers reversed the direction of the current, so that it flowed in the direction of the blue arrows. Under this condition, the circuit diverted the current from the heaters and the chamber was allowed to cool.
Experimental Results
The data curves plotted in Figure 5 are the dark currents measured for the Si-based FDS1010, the Ge-based FDG50, the GaP-based FGAP71, and the InGaAs-based FGA10. Data were acquired continuously while the temperature was between 25 °C and 55 °C (shaded region of Figure 6). Figure 6 is representative of the temperature measured at the photodiode during the experiment. The temperature profile included an initial segment of increasing temperature, followed by a soak that allowed the photodiode to reach the maximum temperature, and concluded with a cool-down. The data plotted in Figure 5 include all dark current measurements measured between 25 °C and 55 °C; current measurements taken at the same temperature, but at different times, overlay one another for each diode.
The data curves plotted in Figure 5 show that the measured dark current magnitudes differed depending on the material composing the photodiode. From lowest to highest:
- GaP-Based Detector FGAP71 (Lowest Dark Current)
- InGaAs-Based Detector FGA10
- Si-Based Detector FDS1010
- Ge-Based Detector FDG50 (Highest Dark Current)
These span approximately 6 orders of magnitude. In all cases, the dark current increased with the temperature of the photodiode, as expected. The individual points on this graph, plotted as diamonds, are the values of the dark current specified for each detector at 25 °C. These points specify a maximum value of dark current at 25 °C; each diode's dark current must be equal to or less than this value at 25 °C, but the dark current may exceed this specification at higher temperatures, as is the case for all but the measured FDS1010 detector.

Click to Enlarge
Figure 5: Dark Current Data Measured for Four Unmounted Photodiodes
The discrete data points, plotted as diamonds, are values of the dark current specified for each detector at 25 °C.

Click to Enlarge
Figure 6: Temperature of a Representative Photodiode Controlled by the Conditions in the Environmental Chamber
Dark current measurements were acquired between 25 °C and 55 °C (indicated by the shaded region) and are plotted in Figure 5 .
Experimental Limitations
Measurements were performed using a single representative of each detector type, as these data were intended to illustrate overall trends. These data should not be taken as specific for a particular diode. The measured dark current is a function of voltage bias, temperature dependence of resistive loads, and other effects. Efforts were made to suppress their influence on these measurements, including using the Keithley 6487 ammeter to provide the required 5 V reverse bias. Using the ammeter removed the need to use a load resisitor, which may exhibit its own temperature dependence. The thermistor was placed as close to the semiconductor sensor as was possible, but it was not placed in direct contact with the sensor. Because of this, there may have been a difference between the measured temperature and the temperature of the semiconductor material. The dark current was measured while the temperature of the environmental chamber was continuously varied. Humidity was not controlled during this experiment.
[1] J. Liu, Photonic Devices. Cambridge University Press, Cambridge, UK, 2005
Noise Equivalent Power (NEP) as a Function of Temperature
Noise Equivalent Power (NEP) was determined as a function of temperature for several unmounted photodetectors. As is described in the following section, NEP is a common metric used to describe the minimum sensitivity of a photodetector. Measurements were taken for silicon (Si), germanium (Ge), gallium phosphide (GaP), and indium gallium arsenide (InGaAs) reverse-biased photodiodes over temperatures from 25 °C to approximately 55 °C.
Noise Equivalent Power
The most general definition of NEP is "the input signal power that results in a signal-to-noise ratio (SNR) of 1 in a 1 Hz output bandwidth." [1] Therefore, in order to determine the NEP, the minimum noise of the photodiode must be determined. When the optical signal is blocked, noise is still present, which is generated by the detector itself. There are two main contributors to photodiode noise: the shot noise due to dark current and thermal noise due to the shunt resistance.
Dark current is a relatively small electric current present in photosensitive devices that flows through the device, including when there is no incident light. More information on the dark current can be found in the "Dark Current as a Function of Temperature" section above. Shot noise is due to the quantized nature of the charge carriers. For the case where there is no light incident on the detector, it can be calculated from the dark current of the detector using the equation [2]
where is is the shot noise, Id is the dark current, q is the electron charge, and fBW is the bandwidth, which will be set to 1 Hz to allow for comparison between different photodiodes.
Thermal, or Johnson, noise is due to the random thermal motion of the charge carriers. Thermal noise will be generated only by the resistive elements of the system. For the photodiode detector, the shunt resistance needs to be considered. Shunt resistance is the resistance of the zero-biased photodiode p-n junction; put another way, it is the inverse of the slope of the I-V curve at the zero-voltage point. Since it is difficult to correctly calculate the slope at the zero crossing, the generally accepted industry practice is to measure the current at V = ±10 mV and then calculate the slope. Then the thermal noise due to the shunt resistance, RSH, can be expressed as:
where it is the thermal noise (expressed as a current), kB is Boltzmann's constant, T is temperature, RSH is shunt resistance, and fBW is the bandwidth.
The total noise, itotal, is the quadrature sum of all noise sources:
Note that this result is the total noise expressed for the current output of the photodiode, while the NEP is expressed in terms of incident optical power. Thus, to compare with the NEP specifications a typical responsivity value,, is chosen for the specified wavelength:
Also, to allow for comparison between different diodes, we set the bandwidth to 1 Hz for the calculation.
Experiment: Dark Current and Shunt Resistance Measurements
NEP was determined over 25 to 55 °C for four representative unpackaged photodiodes: the Si-based FDS1010, the Ge-based FDG50, the GaP-based FGAP71 (previous generation), and the InGaAs-based FGA10.

Click to Enlarge
Figure 1: The nested metal box test fixture with covers removed and FGA10 installed.
Black insulating foam lines the interiors of both boxes.
A: Thermistor; B: FGA10; C: BNC-to-BNC Feedthrough; D: Outer Box; E: Inner Box; F: BNC-to-Triax Feedthrough; G: BNC-to-BNC Feedthrough

Click to Enlarge
Figure 3: An electrical circuit that included a resistive foil heater, TED8040 temperature controller, and two rectifying diodes controlled current flow through the resistive current heater as described in the text.

Click to Enlarge
Figure 2: The Test Setup
The custom-built temperature chamber is on the top of the cart, and the temperature control instrumentation that includes the PRO8000 and TED8040 cards is on the cart's lower shelf.
Experimental Setup
The experimental setup was designed to ensure a constant reverse-bias voltage across the photodiode, control the temperature of the detector, block light from reaching the detector, and establish an electrical path between the detector and the Keithley 6487 ammeter that isolated the measured current from extraneous electromagnetic interference (EMI) noise sources. Dark currents for these detectors can be on the order of nA, which made it important to control these conditions to ensure accurate measurements.
The detector enclosure consisted of a pair of nested aluminum metal boxes, which are shown with their covers off in Figure 1. Only one box would be required to create a light-tight environment for the photodiode, but an outer box was included in the setup to shield the inner box from EMI. The detector was placed inside the inner box, and it was electrically connected to the Keithley 6487 ammeter. The ammeter provided the required reverse-bias voltage and received the output current signal. The electrical signals between the ammeter and the photodiode were routed through the two boxes using a combination of cables and feedthroughs. The bias voltage was connected to the photodiode using coax cables and BNC-to-BNC feedthroughs in the walls of both metal boxes. The current signal from the photodiode was connected to the BNC end of a BNC-to-Triax feedthrough integrated into the wall of the inner box. The signal was then routed through the outer box and to the Keithley 6487 using triax cables and a Triax-to-Triax feedthrough in the outer box. Triax cables were used because coax cables are poorly shielded, and using a coax cable to route the signal to the ammeter could introduce noise from EMI sources to the signal read from the detector. As the outer box shields the inner box from EMI, the noise introduced on the signal as it travels from the detector to the BNC-to-Triax feedthrough is mitigated.
For dark current measurements, the bias voltage was set to 5 V. In order to calculate the shunt resistance, measurements of the current were made with the bias voltage set to +10 mV and -10 mV.
Thermistors were used to monitor the temperatures of each photodiode continuously during the experiment. A piece of thermal tape was used to hold the thermistor flush against the TO cans of the FDG50, FGAP71, and the FGA10 during testing. The photosensor of the FDS1010 is mounted on a ceramic substrate, and when it was tested the thermistor was taped to the back of the substrate. The electrical connection between the thermistor and TSP01 temperature logger was performed using BNC cables, BNC-to-BNC bulkhead feedthroughs to route the signal out of the nested boxes, and a custom BNC-to-phono jack cable to connect to the temperature logger.
A custom temperature chamber based on a XE25C9 standard enclosure was used to conduct these tests. It is shown on top of the cart in Figure 3. The enclosure had a floor and four walls, and insulation was attached to all inner and outer surfaces. A lid for the enclosure was fashioned from a sheet of hardboard bordered with XE25 rails, and insulation was attached to the inner surface of the hardboard. Six resistive foil heaters were affixed to the walls of the XE25C9 enclosure, and they were driven using the PRO8000 chassis fitted with six TED8040 thermoelectric cooler (TEC) controller cards placed on the bottom shelf of the cart in Figure 2. Each TED8040 was interfaced with a heater and a thermistor installed in the chamber. The reading from the thermistor determined the current sent to drive the heater. The chamber was not actively cooled; cooling was instead performed by withdrawing the current driving the heaters and, as an option, opening the lid of the chamber.
The TED8040 units are designed to be interfaced with TECs, which produce heat when current flows in one direction, and which provide cooling when the current flows in the opposite direction. Because of this, when the enclosure exceeded the setpoint temperature, the TED8040 units in this experimental setup did not cut off the driving current. They instead reversed the current flow in an effort to produce cooling. In contrast to TECs, resistive foil heaters generate heat regardless of the direction of current flowing through them. In order to divert the driving current from the heaters, an electrical circuit, diagrammed in Figure 3, that included two rectifying diodes was designed and implemented. Rectifying diodes allow current to flow only in one direction. When the temperature reading from the thermistor was lower than the setpoint temperature, current flowed in the direction of the red arrows and through the resistive heater, and heat was generated. When the temperature of the enclosure exceeded the setpoint temperature, the TED8040 controllers reversed the direction of the current, so that it flowed in the direction of the blue arrows. Under this condition, the circuit diverted the current from the heaters and the chamber was allowed to cool.
Experimental Results
The data curves plotted in Figure 4 are the dark currents measured for the Si-based FDS1010, the Ge-based FDG50, the GaP-based FGAP71, and the InGaAs-based FGA10. Figure 5 shows the calculated shunt resistance values for the same diodes as Figure 4. Data were acquired continuously while the temperature was between 25 °C and 55 °C (shaded region of Figure 7). Figure 7 is representative of the temperature measured at the photodiode during the experiment. The temperature profile included an initial segment of increasing temperature, followed by a soak that allowed the photodiode to reach the maximum temperature, and concluded with a cool-down. The data plotted in Figures 4 and 5 include all dark current measurements measured between approximately 25 °C and 55 °C; current measurements taken at the same temperature, but at different times, overlay one another for each diode.
As shown in Figure 5, the shunt resistance for the measured GaP, InGaAs, and Si detectors is relatively large; therefore in many situations it can be ignored. For the Ge-based FDG50 the shunt resistance is relatively low, especially at higher temperatures. For this diode the shunt resistance might need to be considered if there is a high load involved.
Figure 6 shows the calculated NEP vs. temperature for each diode. Since the NEP is a function of responsivity, which varies with wavelength, the NEP values are calculated at the peak responsivity value as given in the table to the right.
Responsivity Used for NEP Calculation | |
---|---|
FGA10 | 1.05 A/W @ 1550 nm |
FDG50 | 0.85 A/W @ 1550 nm |
FGAP71 | 0.12 A/W @ 440 nm |
FDS1010 | 0.725 A/W @ 970 nm |
The data curves plotted in Figure 6 show that the calculated NEP magnitudes differed depending on the material composing the photodiode. From lowest to highest:
- GaP-Based Detector FGAP71 (Lowest NEP)
- InGaAs-Based Detector FGA10
- Si-Based Detector FDS1010
- Ge-Based Detector FDG50 (Highest NEP)
These span approximately 6 orders of magnitude. In all cases, the dark current increased with the temperature of the photodiode, as expected. The individual points on this graph, plotted as diamonds, are the values of the dark current specified for each detector at 25 °C. These points specify a maximum value of dark current at 25 °C; each diode's dark current and NEP must be equal to or less than this value at 25 °C, but the dark current and NEP may exceed this specification at higher temperatures.

Click to Enlarge
Figure 4: Dark Current Data Measured for Four Unmounted Photodiodes
The discrete data points, plotted as diamonds, are values of the dark current specified for each detector at 25 °C.

Click to Enlarge
Figure 5: Shunt Resistance Calculated as Described in the Text for Four Unmounted Photodiodes

Click to Enlarge
Figure 6: NEP Calculated from the Quadrature Sum of Shot Noise and Thermal Noise for Four Unmounted Photodiodes
The discrete data points, plotted as diamonds, are values of the NEP specified for each detector at 25 °C. There is no point for FGA10 since the NEP is not specified at the peak responsivity wavelength.

Click to Enlarge
Figure 7: Temperature of a Representative Photodiode Controlled by the Conditions in the Environmental Chamber
Dark current measurements were acquired between 25 °C and 55 °C (indicated by the shaded region) and are plotted in Figure 5 .
Experimental Limitations
Measurements were performed using a single representative of each detector type, as these data were intended to illustrate overall trends. These data should not be taken as specific for a particular diode. The measured currents were functions of voltage bias, temperature dependence of resistive loads, and other effects. Efforts were made to suppress their influence on these measurements, including using the Keithley 6487 ammeter to provide the required 5 V reverse bias. Using the ammeter removed the need to use a load resistor, which may exhibit its own temperature dependence. The thermistor was placed as close to the semiconductor sensor as was possible, but it was not placed in direct contact with the sensor. Because of this, there may have been a difference between the measured temperature and the temperature of the semiconductor material. The currents were measured while the temperature of the environmental chamber was continuously varied. Humidity was not controlled during this experiment.
[1] Thorlabs' Noise Equivalent Power White Paper.
[2] Quimby, Richard S. Photonics and Lasers: An Introduction. Wiley-Interscience, Hoboken, NJ, 2006, pp 241-244.
Beam Size and Photodiode Saturation

Click to Enlarge
Figure 1: Normalized change in output current for a fixed incident power as the photodiode Device Under Test (DUT), FDS1010, was translated through the focus of a lens.
We present laboratory measurements showing the effect of beam size on the saturation point of a Thorlabs silicon photodiode. As defined here, saturation is a 1% deviation from the linear response region. As illustrated in Figure 1, the photodiode saturated at lower incident power levels as the beam size decreased. Several additional calculations and experiments were performed to verify the cause of the change in saturation was not a function of the power density. These results suggest that users should be cognizant of the beam size when attempting to measure absolute power with a power sensor, such as our S130C photodiode power sensor.
For our experiment, we used the SM1PD1A mounted photodiode, which consists of an FDS1010 photodiode in an SM1-threaded housing. An 830 nm superluminescent diode was used as the light source. A beamsplitter directed 20% of the light to a monitor photodiode while the remainder was transmitted through a focusing lens. Power values were calibrated using an integrating sphere, while the beam size of the focusing beam was calibrated with a beam profiler mounted to a translation stage. After calibration, the beam profiler was replaced with the device under test (DUT), an SM1PD1A at a 0 V bias. The output current was measured with an ammeter to remove the need for a load resistor. We then recorded datasets as the beam diameter was continuously scanned from 0.06 mm to 5 mm while power was held constant, as well as measurements for beam diameters ranging from 1 mm to 5 mm (measured to the 5% clip level) as the incident power was continuously scanned from 0.12 mW to 5 mW.
The figures show the results from the measurements. Figure 1 (right) and Figure 2 (below) show the deviation from linear response for continuously varying beam diameter at a fixed 1 mW incident power (Figure 1) and continuously varying incident power for several beam diameters (Figure 2). Figure 1 shows that for a 1 mW input, the photodiode saturated at beam sizes less than 300 µm. Figure 2 shows that beam diameters ≥2 mm did not saturate at the power levels investigated.
One hypothesis for these results was that local saturation due to a large power density locally depleted or reduced the population of available carriers. In Figure 3, we calculated the power density by taking the quotient of the measured power and beam diameter presented in Figure 2 and plotted the current output as a function of that power density. If the results were due to local saturation, we would have expected that all of the beam diameters would saturate at a single power density; however, this was not the case.
Since the results presented in Figure 3 coupled the beam size change with a change in optical power density, we performed another experiment to increase the power density incident on the sensor within the same envelope of beam area to see if there was a change in the saturation point. This was done by segmenting the Gaussian beam into an array of beamlets with a microlens array, concentrating the overall optical power into smaller spots within the same Gaussian envelope. This created larger power densities while maintaining a similar electrical path to the sensor leads. Figure 4 shows the same results as Figure 2 with the results from the microlens array overlaid as dashed lines. Since the results are nearly identical to the original Gaussian beam for all diameters, the saturation appears to be dependent on the overall beam diameter and independent of the power density. In the complete Lab Facts presentation, we discuss how these results support the theory of Scholze et al. that the change in saturation with beam size is due to a change in the series resistance of the photodiode [1].

Click to Enlarge
Figure 2: Percent Deviation from the linear response with increasing power for 1 mm - 5 mm beam diameters. The 1% deviation level is indicated with a dashed horizontal line.

Click to Enlarge
Figure 3: Normalized output current versus power density for 1 mm - 5 mm beam diameters. This shows the saturation effects do not appear to be based on a single power density.

Click to Enlarge
Figure 4: Normalized output current versus optical power for 2 mm - 5 mm beam diameter with (Dashed Line) and without (Solid Line) the microlens array (MLA) prior to the photodiode.
[1] F. Scholze, R. Klein, R. Muller, Linearity of silicon photodiodes for EUV radiation. 2004 Proc. SPIE 5374 926–34.
Effective Reverse Bias Voltage as a Function of Incident Optical Power
Introduction
The bandwidth (and rise time) of a photodiode is known to be a function of the effective bias across it. Therefore, the effective bias must be tuned in response to the generated photocurrent from the optical power incident on the diode to maintain the desired bandwidth. This lab fact investigates the relationship between the effective reverse bias voltage across a photodiode and the CW optical power incident on it in order to create a reliable model of a biased photodiode.
Circuit Analysis
To begin creating this model, the photodiode circuit shown in Figure 2 to the right is examined using Ohm’s law and assuming a constant DC voltage source; this yields the equation
Veff = V0 - iPD * (RP + RL).
(1)
The effective bias voltage (Veff) across the photodiode equals the initial voltage from the source (V0) minus the product of the photocurrent (iPD) and the sum of the resistance of the bias module's resistor (RP) and the load resistor (RL).
To find how Veff changes with respect to the incident optical power (P), iPD is replaced in the above equation with its definition as the product of the wavelength-dependent responsivity [ℜ(λ)] of the photodiode and P:
iPD = ℜ(λ) * P.
(2)
Using Ohm’s law on the load resistor such that iPD = VL / RL, the equation above was rewritten to solve for ℜ(λ):
ℜ(λ) = VL / (P * RL ).
(3)
Assuming that the voltage drop across the load resistor (VL) is linear with respect to increasing P, the ratio of the change in VL and P can be established as the ratio:
m = Δ VL / Δ P.
(4)
These equations can be combined to form:
Veff = V0 - (m / RL) * P * (RP + RL).
(5)
The ratio m can be calculated from empirical measurements of VL and P for each photodiode, allowing this equation to be used as a model for how Veff changes with respect to P.
Experiment
An experiment was developed to measure the change in VL with respect to changes in P. Output from a fiber-coupled laser diode was collimated, passed through a neutral density filter, and then focused onto the Device Under Test (DUT) via an off-axis parabolic mirror. A photograph of the setup used can be seen in Figure 1 to the upper right. Neutral density filters with a range of optical densities were used to vary the power incident on the photodiode. For each photodiode used, the distance between the sensor surface and focusing mirror was adjusted so that the spot size was approximately half the size of each photodiodes’ detector area. An oscilloscope was used to measure V0, VL, and the sum Veff + VL. A multimeter was used to measure the effective bias directly.
Results
VL was plotted against P for each DUT; an example of the results for the silicon photodiode can be seen in Figure 3 to the right. A linear trend line was created for each data set, and the slope of that line corresponds to m in Equation 4. This value can be used in Equation 5 to calculate the effective bias voltage. Then, Veff can be plotted with respect to the incident power and compared to the measured values from the oscilloscope. These results are shown in the three graphs displayed in Figure 4 below. The model was found to be consistent with measured experimental results, which showed that Veff decreases as P increases (with V0, RP, and RL held constant). This effect is significant, because the voltage set at the voltage source is not necessarily the bias voltage applied to the photodiode; one must account for resistive components within the circuit to calculate the effective bias voltage applied across the photodiode.

Figure 1: This setup was used in the experiment in order to verify our model of the effective voltage across the photodiode.

Click to Enlarge
Figure 2: Circuit Diagram of Biased Photodiode
_Plot_G1-300.gif)
Click to Enlarge
Figure 3: A single voltage vs. incident power graph used to find m for the final model, Equation 5.
About Our Lab Facts
Our application engineers live the experience of our customers by conducting experiments in Alex’s personal lab. Here, they gain a greater understanding of our products’ performance across a range of application spaces. Their results can be found throughout our website on associated product pages in Lab Facts tabs. Experiments are used to compare performance with theory and explore the benefits and drawbacks of using similar products in unique setups, in an attempt to understand the intricacies and practical limitations of our products. In all cases, the theory, procedure, and results are provided to assist with your buying decisions.
Pulsed Laser Emission: Power and Energy Calculations
Determining whether emission from a pulsed laser is compatible with a device or application can require referencing parameters that are not supplied by the laser's manufacturer. When this is the case, the necessary parameters can typically be calculated from the available information. Calculating peak pulse power, average power, pulse energy, and related parameters can be necessary to achieve desired outcomes including:
- Protecting biological samples from harm.
- Measuring the pulsed laser emission without damaging photodetectors and other sensors.
- Exciting fluorescence and non-linear effects in materials.
Pulsed laser radiation parameters are illustrated in Figure 1 and described in the table. For quick reference, a list of equations are provided below. The document available for download provides this information, as well as an introduction to pulsed laser emission, an overview of relationships among the different parameters, and guidance for applying the calculations.
Equations: |
||||
![]() |
and | ![]() |
||
![]() |
||||
![]() |
||||
![]() |
||||
Peak power and average power calculated from each other: |
||||
![]() |
and | ![]() |
||
Peak power calculated from average power and duty cycle*: | ||||
![]() |
*Duty cycle (![]() |

Click to Enlarge
Figure 1: Parameters used to describe pulsed laser emission are indicated in the plot (above) and described in the table (below). Pulse energy (E) is the shaded area under the pulse curve. Pulse energy is, equivalently, the area of the diagonally hashed region.
Parameter | Symbol | Units | Description | ||
---|---|---|---|---|---|
Pulse Energy | E | Joules [J] | A measure of one pulse's total emission, which is the only light emitted by the laser over the entire period. The pulse energy equals the shaded area, which is equivalent to the area covered by diagonal hash marks. | ||
Period | Δt | Seconds [s] | The amount of time between the start of one pulse and the start of the next. | ||
Average Power | Pavg | Watts [W] | The height on the optical power axis, if the energy emitted by the pulse were uniformly spread over the entire period. | ||
Instantaneous Power | P | Watts [W] | The optical power at a single, specific point in time. | ||
Peak Power | Ppeak | Watts [W] | The maximum instantaneous optical power output by the laser. | ||
Pulse Width | ![]() |
Seconds [s] | A measure of the time between the beginning and end of the pulse, typically based on the full width half maximum (FWHM) of the pulse shape. Also called pulse duration. | ||
Repetition Rate | frep | Hertz [Hz] | The frequency with which pulses are emitted. Equal to the reciprocal of the period. |
Example Calculation:
Is it safe to use a detector with a specified maximum peak optical input power of 75 mW to measure the following pulsed laser emission?
- Average Power: 1 mW
- Repetition Rate: 85 MHz
- Pulse Width: 10 fs
The energy per pulse:
seems low, but the peak pulse power is:
It is not safe to use the detector to measure this pulsed laser emission, since the peak power of the pulses is >5 orders of magnitude higher than the detector's maximum peak optical input power.
Posted Comments: | |
Malcolm Higman
 (posted 2021-01-18 10:11:54.67) Your data-sheet has no dimensions on the pin positions which appear to be non-standard. Do you have a drawing with the pin positions with dimensions? Thanks. Malcolm Ben Garber
 (posted 2020-05-27 17:20:24.69) I was wondering what the window thickness is on the FDS015 (for lens matching--I'm considering the N414TM-B or C392TME-B). YLohia
 (posted 2020-05-28 04:29:17.0) Thank you for contacting Thorlabs. The window thickness for this photodiode is ~0.21 mm. user
 (posted 2020-04-23 11:46:32.8) You should offer the extended range InGaAs detectors in a premounted configuration. asundararaj
 (posted 2020-04-23 02:06:42.0) Thank you for your valuable feedback. I have posted in our internal engineering forum about extending the line of photodiodes we offer as mounted photodiodes for future consideration. Haixuan Lin
 (posted 2020-02-14 10:35:12.473) Please help to check the angle dependence of FDS1010.
If the diode emitting a cone up to 20deg in the fast axis, will it affect the responsivity of FDS1010? asundararaj
 (posted 2020-02-17 10:01:36.0) Than you for contacting Thorlabs. When using a photodiode to detect a diverging beam, the detection would depend on the amount of light hitting the photodiode as well as the spatial uniformity of the diode. You can find the Spatial Uniformity of this and other diodes in the Lab Fact linked below. In addition, the FDS1010 has a broadband AR Coating on the active area. From this coating, there are some losses at higher angles of incidence due to Fresnel reflections. I will contact you via email about the angular dependence of the measured signal. Lab Fact - https://www.thorlabs.com/newgrouppage9.cfm?objectgroup_id=10741&tabname=Spatial%20Uniformity Ben Garber
 (posted 2019-11-19 15:06:07.05) Dear Thorlabs,
Looking at the specs for the FDS02, it's unclear whether the responsivity is with respect to the power in the fiber or incident power. Is the responsivity defined with respect to the power in the fiber? If not, what is the coupling efficiency? asundararaj
 (posted 2019-11-21 08:58:37.0) Thank you for contacting Thorlabs. The responsivity is given in terms of the power incident on the photodetector. We typically expect a coupling loss of 10% and can vary marginally based on alignment. anais leproux
 (posted 2019-11-14 11:17:19.773) Hello,
I could not find the value of the shunt resistance of the photodiodes FDS100 and FD11A. Would it be possible to have them please?
Thank you,
Anais Leproux YLohia
 (posted 2019-11-20 04:26:06.0) Hello Anais, thank you for contacting Thorlabs. The shunt resistances are 1 G Ohm (FDS100) and 5-100 G Ohm (FD11A) at room temperature. Please note that this parameter is not screened for individual units and can vary significantly. Scott Hunter
 (posted 2019-11-12 13:07:10.057) Hello, I am considering this product in my design. Can you please let me know where I can get more detailed information on this product such as performance specifications with 0V bias? Thank you! YLohia
 (posted 2019-11-12 02:34:33.0) Hello, thank you for contacting Thorlabs. The Dark Current and Capacitance values are given at various bias levels, and can be accessed by clicking on the blue "info" icon in the specs table. dayana A
 (posted 2019-10-24 19:25:00.727) Please share the Maximum Forward Current of FGA01FC YLohia
 (posted 2019-10-24 10:44:04.0) The maximum forward current of the FGA01FC is 5 mA. Tariq Shamim Khwaja
 (posted 2019-10-08 13:55:26.557) What is the maximum input power density (damage threshold) for Ge-photodiodes? FDG10X10 asundararaj
 (posted 2019-10-09 10:43:06.0) Thank you for contacting Thorlabs. We do not have estimate on the damage threshold for the photodiodes. We typically recommend keeping the output current to be <10mA to avoid the internal wire in the FDG10x10 from failing. You can estimate the output current for your input power and wavelength from the responsivity graph on the 2nd page of the spec sheet at https://www.thorlabs.com/_sd.cfm?fileName=TTN126580-S01.pdf&partNumber=FDG10X10 user
 (posted 2019-08-02 09:08:10.89) What is the series resistance of the photodidoe FDS015 and FDS025? The reason behind this is the impedance matching between the output impedance of the photodiode and the input impedance of a transimpedance amplifier. YLohia
 (posted 2019-08-16 02:34:12.0) Hello, thank you for contacting Thorlabs. The series resistance for both of these is on the order of 50 - 100 Ohm typically. Andrey Kuznetsov
 (posted 2019-07-13 17:22:48.15) Some of these photodiodes look like a Hamamatsu product, Hamamatsu distinguishes some products as Si Photodiode and others as Si PIN Photodiode. Hamamatsu's offerings for InGaAs is exclusively for InGaAs PIN Photodiodes. Thorlabs does not distinguish photodiodes as whether they are PN or PIN. Can you explain what the effect on the specifications will be if a PIN photodiode is used in a zero bias photovoltaic mode? None of Thorlab's InGaAs are listed as PIN, and I was unable to find any vendor that sells a non-PINed InGaAs photodiode, so Thorlabs must be selling PINed photodiodes. Shouldn't PINed photodiodes be biased for they to work as specified, or will they still work fine like a regular photodiode, just slower rise/fall time and lower dark current than listed? What about saturation limit? asundararaj
 (posted 2019-07-19 04:57:52.0) Without a bias voltage, the photodiode will have a much larger capacitance which leads to slower rise/fall time. Dark current spec however will not be listed under a Photovoltaic mode, because by definition of dark current, it requires a bias voltage (hence, this is sometimes specified under a very small voltage, e.g.10mV). The NEP in the photovoltaic mode would normally only accounts the noise from the shunt resistance. So it will be different as well. Our NEP spec accounts both of the Johnson noise from the shunt resistance and the shot noise from the dark current. As for the saturation power, it would decrease at 0 V Bias. (https://www.thorlabs.com/newgrouppage9.cfm?objectgroup_id=10741)
Other parameters that we do not specify can also change with and without a Bias Voltage. For instance, adding a Bias Voltage would theoritically improve linearity. Another parameter that would change is the responsivity at various wavelengths. The amount of variance would vary from diode to diode.
The InGaAs detectors that we carry are all PIN diodes. Some of our Si diodes are also PIN diodes. At higher wavelengths, the penetration depth is much larger so adding the intrinsic layer to the diode improves the diodes performance. However, in that case these photocurrents would be from diffusion and hence, its speed is much slower. user
 (posted 2019-05-21 09:07:54.173) Hi,
I would like to use FDS100 Photo diode for Data communication application.
I would like to know the Pixel Size and format of FDS 100.
Please share the same at the earliest possible.
Regards YLohia
 (posted 2019-05-21 08:44:32.0) Hello, the FDS100 is a silicon photodiode (not a CCD/CMOS camera), and thus, does not have a pixel size or format. That being said, it has an active area of 3.6 mm x 3.6 mm. If you are interested in cameras, please see our selection here : https://www.thorlabs.com/navigation.cfm?guide_id=2025. user
 (posted 2018-10-28 13:49:13.483) Sorry,the last question I asked was sensitivity map, not responsivity plots, I still cannot find out what is the sensitivity range(in dBm). YLohia
 (posted 2018-12-27 03:59:01.0) Hello, thank you for the clarification. This information can be found in our "Lab Facts" tab above, under "Photodiode Spatial Uniformity". user
 (posted 2018-10-25 22:01:07.26) Hello! I want to know what is the sensitivity map of this photodiode?or what factor can take place of sensitivity? YLohia
 (posted 2018-10-26 08:57:44.0) The responsivity plots can be found on the spec sheet or by clicking the blue "info" icon on the table. dylan
 (posted 2018-09-05 14:10:05.543) Hi, is the pinout diagram for FGA01 viewed from the bottom? The pinout for FGA01FC explicitly states the view is from the bottom, and I believe the photodiodes are identical, but I need confirmation that this is the case.
Kindest regards, nbayconich
 (posted 2018-09-06 03:12:42.0) Thank you for contacting Thorlabs. Yes that is correct, both of these photodiodes have identical pin configurations. Our pin configurations will typically show a bottom view of the photodiode housing. donyakhaledyan
 (posted 2018-07-16 03:49:37.237) hello I have a question and the answer of my question
is so necessary so thank you so much if you help me.
for these detectorsfds10x10&fds1010)What is the proper interface? YLohia
 (posted 2018-07-23 04:44:41.0) Hello, thank you for contacting Thorlabs. I'm not sure I understand what you mean by "what is the proper interface?". Are you asking if these detectors are fiber coupled? If you're asking about the packaging type (e.g., TO-5, etc.), unfortunately, the FDS10X10 and FDS1010 don't have a standard. I have reached out to you directly to discuss this further. lebouquj
 (posted 2018-04-19 16:33:12.957) Hi, Do you sell the fiber bulkhead of FGA01FC alone (without the diode). I cannot find it in your website. Thanks, Jean-Baptiste YLohia
 (posted 2018-04-20 10:56:39.0) Hello Jean-Baptiste, thank you for contacting Thorlabs. I will reach out to you directly to discuss the possibility of offering this. user
 (posted 2017-07-17 15:09:46.207) I am seeing ~10x increase in responsivity for FDS010 with a reverse biased circuit like you have in your tutorial in Fig. 2. I am using 12VDC, with 1k resistor in filter, and 10k load resistor. Is this expected, or am I doing something wrong. tfrisch
 (posted 2017-08-09 05:35:48.0) Hello, thank you for contacting Thorlabs. While I would not expect the responsivity to change with bias voltage, increasing the bias will increase the range over which the diode will have linear response between input power and output current before saturating. If you would like to discuss this application further, please reach out to us at TechSupport@Thorlabs.com. marty.lawson
 (posted 2017-02-01 12:49:31.84) How large of a fiber optic core can the the FDS02 photo-diode be used with before coupling efficiency drops? tfrisch
 (posted 2017-02-16 02:14:08.0) Hello, thank you for contacting Thorlabs. While the active area is 250um in diameter, the largest fiber we have that is smaller than that would be a 200um core. The FDS02 does not include a ball lens between the fiber and the detector. I will reach out to you with more details. faryads
 (posted 2016-03-22 21:36:37.053) Hi, is the FGA01FC a multi-quantum-well structure photodiode? besembeson
 (posted 2016-03-25 12:26:10.0) Response from Bweh at Thorlabs USA: The FGA01FC is not a multi-quantum-well structure. This is simply a doped InGaAs material to create a P-N junction for charge transfer when illuminated by light of suitable wavelength. Quantum wells on the other hand are heterostructure made by joining materials, in layers at the atomic level, which typically leads to an emission. akpabioubongabasi
 (posted 2016-02-29 14:16:38.723) Please I need a Photodector with wide sensing area, a wavelength of 650nm and which comes with an SMA Connector besembeson
 (posted 2016-03-09 12:16:53.0) Response from Bweh at Thorlabs USA: The SM05PD1A (Large Area Mounted Silicon Photodiode, 350-1100 nm, Cathode Grounded) could be a suitable recommendation. Others can be found at the following link on our website: https://www.thorlabs.com/newgrouppage9.cfm?objectgroup_id=1285 user
 (posted 2015-10-16 14:16:32.147) What is the series and shunt resistance of FDS100? besembeson
 (posted 2015-10-27 04:54:33.0) Response from Bweh at Thorlabs USA: We don't have the exact values from the manufacturer of the photodiode but the shunt resistance should be in the Giga Ohm range while the series resistance should be extremely low that it can be negligible. ehrler
 (posted 2015-09-24 03:41:03.583) Do you offer calibration for the DSD2 photodiode?
Thanks! besembeson
 (posted 2015-10-08 12:06:35.0) Response from Bweh at Thorlabs USA: This is not possible for the DSD2. There is a limitation to the minimum detector size for us to do such calibration, which is 2mm minimum. The InGaAs sensor is only about 1.5mm. jarkko.piirto
 (posted 2015-03-24 10:27:03.187) I would need a photodiode set up with specific mount (3pcs) for picosecond laser alignment. Focused spot size (1/e2) is <100µm. Succesfull guidance will mean purchase.. jlow
 (posted 2015-03-24 04:42:43.0) Response from Jeremy at Thorlabs: We will need more information on your application first. We will contact you directly about this. aklossek
 (posted 2014-05-09 07:48:19.477) Dear ladies and gentlemen,
I am looking for a Si photodiode for a stimulated raman microscope, where the modulation of a ps-laser beam must be detected. The diode has to work in MHz range, quite large active area and must be sensitive between 800 and 1000nm. Up to now I thought about the DET100A.
But now I have doubts that it will saturate to fast. Can you suggest another diode, which saturates at high powers to detect the laser beam.
Best regards
André Klossek jlow
 (posted 2014-05-13 08:43:47.0) Response from Jeremy at Thorlabs: I will contact you directly to get more information on your application and make a recommendation. tanzwei
 (posted 2013-05-05 06:39:49.27) Could you like to tell me the damage threshold of FGA10 ? jlow
 (posted 2013-05-09 11:19:00.0) Response from Jeremy at Thorlabs: I would recommend keeping the output current to be <10mA to avoid the internal wire in the FGA10 from failing. You can estimate the output current for your input power and wavelength from the responsivity graph on the 2nd page of the spec sheet at http://www.thorlabs.com/Thorcat/2200/FGA10-SpecSheet.pdf. jlow
 (posted 2012-10-24 16:14:00.0) Response from Jeremy at Thorlabs: There's a ball lens covering the chip and it is not AR coated. t.schmoll
 (posted 2012-10-18 13:30:14.92) Is there a window or a ball lens covering the chip? Is the window or ball lens AR coated? If so, for which wavelength is it optimized?
Thank you!
Tilman jlow
 (posted 2012-09-05 09:15:00.0) Response from Jeremy at Thorlabs: I will get in contact with you directly to discuss about your application. kkkdane
 (posted 2012-09-03 10:17:41.0) Hi, I’m a researcher in South of Korea
Recently, I am developed infrared moisture detector. And I used LED and PD that is your product.
I have a question.
What is the question PD, LD(laser diode) and LED that use infrared moisture detector or infrared moisture analyzer?
Recently, What other companies use the LED, PD that used the water detector of Commercial products?
And what is commercial product?
And, If you will give a detailed information about the LED, PD.
I appreciate your help. jlow
 (posted 2012-08-23 16:31:00.0) Response from Jeremy at Thorlabs: The junction capacitance between 4V and 5V is pretty much flat. Unfortunately we do not have any data on the capacitance and fall time specs beyond 5V. Mathias.Helsen
 (posted 2012-08-22 06:14:07.0) I would like to use this diode as a detector for microwave modulated light, but the fall time at 5V is too high. How much doe the capacitance and fall time change when the bias voltage is increased? jlow
 (posted 2012-08-17 11:16:00.0) Response from Jeremy at Thorlabs: We are not able to disclose the thicknesses for the PIN layers. cardoza.david
 (posted 2012-08-17 09:50:00.0) Is there a way to get find out the thickness of the p, i and n regions of the FDS010 diodes? Thank you. jlow
 (posted 2012-08-16 13:42:00.0) Response from Jeremy at Thorlabs: I will get in contact with you directly for the Excel spreadsheet. Please note that the spectral responsivity of your photodiode can be quite different than what is shown online. One alternative to using the FDS100 is the FDS100-CAL (http://www.thorlabs.com/NewGroupPage9.cfm?ObjectGroup_ID=2822&pn=FDS100-CAL) which is the NIST calibrated version of the FDS100. brian.cox
 (posted 2012-08-16 12:13:51.0) Is the raw spectral responsivity data for the FDS100 available in an Excel spreadsheet? I'd like to get a more exact A/W value for my specific wavelengths of interest. Thanks! tcohen
 (posted 2012-03-22 14:27:00.0) Response from Tim at Thorlabs: Thank you for your feedback. If the window is removed the diode can be easily damaged and absorb water from the atmosphere. This will be detrimental to the performance of the FGA10. If the window is removed we recommend storing it in an N2 dry box. frank
 (posted 2012-03-22 13:31:41.0) Can you tell me if the performance of the FGA10 will degrade if its window is removed? bdada
 (posted 2012-03-16 12:33:00.0) Response from Buki at Thorlabs:
Thank you for your suggestion. We will work on adding dark current vs bias voltage charts to our website. user
 (posted 2012-03-15 19:02:40.0) Please consider adding a plot that shows the dark current as a function of bias voltage. bdada
 (posted 2012-01-31 23:43:00.0) Response from Buki at Thorlabs:
The temperature does have a slight effect on the responsivity, but mostly in near the bandgap region. We have sent you some typical curves to review. ale.cere
 (posted 2012-01-30 12:27:49.0) I am currently working with a FDG05 and I notice that the efficiency changes with temperature, as indicated also in the datasheet. Is it available any data regarding the expected change in responsivity as funcion of temperature? jjurado
 (posted 2011-07-07 09:57:00.0) Response from Javier at Thorlabs to jasiel.mora: Thank you very much for contacting us! We actually show a recommended circuit diagram for our photodiodes on their spec sheets. Take a look at the spec sheet for the FDG03 here: http://www.thorlabs.com/Thorcat/13800/13846-S01.pdf
I will contact you directly in case you have any further questions. jasiel.mora
 (posted 2011-07-06 16:42:48.0) Could you please sugest any connection diagram for the sensor FDG03?
Thanks. jjurado
 (posted 2011-04-20 12:09:00.0) Response from Javier at Thorlabs to last poster: Thank you very much for contacting us. The shunt resistance of the FDS1010 photodiode is in the range of 50-200 MOhm (@ 10 mV reverse bias). Also, we have added a graph of the maximum, average, and minimum responsivity values for the FDS1010 (See Responsivity Graphs tab). Please contact us at techsupport@thorlabs.com if you have any further questions or comments. user
 (posted 2011-04-20 13:08:43.0) What is the shunt resistance of FDS1010? Its not in the specs. A typ. and min. responsivity value would be useful as well. julien
 (posted 2011-01-18 16:08:51.0) A response from Julien at Thorlabs: The FGA04 can only be calibrated at a predefined fixed wavelength by using a fiber coupled laser source. A calibration over the whole wavelength range of this photodiode is unfortunately not possible due to its small active area. Such a calibration would be made in free space using a monochromator, whose output beam diameter is about 1.5mm. Such a beam would largely overfill the active sensor area, and thus make the calibration highly inaccurate. You can contact our tech support (techsupport@thorlabs.com) to further discuss which solutions could be adapted to your need user
 (posted 2011-01-18 17:46:35.0) why a NIST-traceable calibration is not possible for FGA04? Im looking for a fiber-coupled detector that is provided with calibration. kleap
 (posted 2010-10-28 13:45:47.0) Our FDG05 are failing roughly 3 months of use. What is the expected life of these detectors? We are exposing indirect UV light around 5W/cm2 of intensity to the LADs. Could this be of a concern? Thorlabs
 (posted 2010-10-28 15:01:31.0) Response from Javier at Thorlabs to kleap: at 5 W/cm^2, the detector will most likely be saturated; however, we specify a damage threshold of 10 W/cm^2 for the FDG05, so I do not expect excessive power to be the reason for failure. Also, we do not have a lifetime spec, since there are too many factors involved. I will contact you directly to troubleshoot your application. Thorlabs
 (posted 2010-07-23 14:06:31.0) Response from Javier at Thorlabs to ranutyagi: Thank you for your feedback. With an input of 10 mW, you will most likely end up damaging your photodiodes. As a guideline, we specify a maximum input power density of 100 mW/cm^2. So, for example, if we assume that you have a 10 mW, 2 mm diameter beam at the input, the resulting power density is ~333mW/cm^2, which clearly exceeds the damage threshold. For linear operation of the photodiode, we recommend limiting the input to ~ 1 mW. Above this value, the diode undergoes saturation and, eventually, damage. ranutyagi
 (posted 2010-07-23 07:03:58.0) I am using FDS100 and FDS010 with CW 10mW peak power laser diode. will it be damaging my photodiode? How much is the maximum input power these diodes can sustain. Adam
 (posted 2010-04-29 16:58:35.0) A response from Adam at Thorlabs to marcoc: Saturation occurs for these diodes at approximately 10mW. We would suggest using these diodes with peak and average powers that are less than 10mW if you want to avoid saturation. marcoc
 (posted 2010-04-29 16:51:34.0) Any idea about the saturation for pulsed (50fs) laser beam at 800 nm ?
thanks
marco apalmentieri
 (posted 2010-01-14 15:34:33.0) A response from Adam at Thorlabs to Curtis: The operating and storage temperature ranges for the FDS100 are the following: -25 to +85 deg C operating, -40 to +100 deg C storage. curtis.m.ihlefeld
 (posted 2010-01-14 15:12:09.0) Dear Sirs,
I have several FDS100 photodiodes and would like to know the allowable temperature ranges for operation and storage.
Regards,
Curtis Ihlefeld danhickstein
 (posted 2009-08-07 14:16:56.0) Dear Thorlabs,
It would be nice to have the wavelength response for the FDS02 plotted on the Graphs page. I found the graph on the spec sheet, but it would be nice to see it plotted on the same graph as the rest of the FDS series.
Regards,
Dan Tyler
 (posted 2009-02-02 09:25:34.0) A response from Tyler at Thorlabs to ocarlsson: The FGA04 spec sheet available under the Drawings and Documents tab lists the max forward current as 10 mA and the damage threshold at 70 mW. The damage threshold is the point at which the photodiode sensor will fail, however, internal wires in the FGA04 package will fail when the forward current exceeds 10 mA. Use the responsivity curve in the spec sheet to approximate the forward current for a given wavelength or contact our technical support department for assistance. An optical fiber attenuator like the FA05T, FA10T, FA15T, or FA25T can be used in to reduce the power in the optical fiber to a level that is safe to use with the FGA04. Thank you for your question, I will be adding a note to the bottom of the table on the Specs tab to help future customers with this issue. ocarlsson
 (posted 2009-01-16 02:31:20.0) The FGA04 max current is 10mA and damage threshold is 100mW. Responsivity 0.8.
How is the damage threshold calculated?
Best regards
Olle |
The following table lists Thorlabs' selection of photodiodes and photoconductive detectors. Item numbers in the same row contain the same detector element.
Photodetector Cross Reference | ||||||
---|---|---|---|---|---|---|
Wavelength | Material | Unmounted Photodiode |
Unmounted Photoconductor |
Mounted Photodiode |
Biased Detector |
Amplified Detector |
150 - 550 nm | GaP | - | - | SM05PD7A | DET25K2 | PDA25K2 |
200 - 1100 nm | Si | FDS010 | - | SM05PD2A SM05PD2B |
DET10A2 | PDA10A2 |
Si | - | - | SM1PD2A | - | - | |
320 - 1000 nm | Si | - | - | - | - | PDA8A2 |
320 - 1100 nm | Si | FD11A | SM05PD3A | PDF10A2 | ||
Si | - | - | - | DET100A2 | PDA100A2 | |
340 - 1100 nm | Si | FDS10X10 | - | - | - | - |
350 - 1100 nm | Si | FDS100 FDS100-CAL a |
- | SM05PD1A SM05PD1B |
DET36A2 | PDA36A2 |
Si | FDS1010 FDS1010-CAL a |
- | SM1PD1A SM1PD1B |
- | - | |
400 - 1000 nm | Si | - | - | - | - | PDA015A(/M) FPD310-FS-VIS FPD310-FC-VIS FPD510-FC-VIS FPD510-FS-VIS FPD610-FC-VIS FPD610-FS-VIS |
400 - 1100 nm | Si | FDS015 b | - | - | - | - |
Si | FDS025 b FDS02 c |
- | - | DET02AFC(/M) DET025AFC(/M) DET025A(/M) DET025AL(/M) |
- | |
400 - 1700 nm | Si & InGaAs | DSD2 | - | - | - | - |
500 - 1700 nm | InGaAs | - | - | - | DET10N2 | - |
750 - 1650 nm | InGaAs | - | - | - | - | PDA8GS |
800 - 1700 nm | InGaAs | FGA015 | - | - | - | PDA015C(/M) |
InGaAs | FGA21 FGA21-CAL a |
- | SM05PD5A | DET20C2 | PDA20C2 PDA20CS2 |
|
InGaAs | FGA01 b FGA01FC c |
- | - | DET01CFC(/M) | - | |
InGaAs | FDGA05 b | - | - | - | PDA05CF2 | |
InGaAs | - | - | - | DET08CFC(/M) DET08C(/M) DET08CL(/M) |
PDF10C/M | |
800 - 1800 nm | Ge | FDG03 FDG03-CAL a |
- | SM05PD6A | DET30B2 | PDA30B2 |
Ge | FDG50 | - | - | DET50B2 | PDA50B2 | |
Ge | FDG05 | - | - | - | - | |
900 - 1700 nm | InGaAs | FGA10 | - | SM05PD4A | DET10C2 | PDA10CS2 |
900 - 2600 nm | InGaAs | FD05D | - | - | DET05D2 | - |
FD10D | - | - | DET10D2 | PDA10D2 | ||
950 - 1650 nm | InGaAs | - | - | - | - | FPD310-FC-NIR FPD310-FS-NIR FPD510-FC-NIR FPD510-FS-NIR FPD610-FC-NIR FPD610-FS-NIR |
1.0 - 5.8 µm | InAsSb | - | - | - | - | PDA10PT(-EC) |
2.0 - 5.4 µm | HgCdTe (MCT) | - | - | - | - | PDA10JT(-EC) |
2.0 - 8.0 µm | HgCdTe (MCT) | VML8T0 VML8T4 d |
- | - | - | PDAVJ8 |
2.0 - 10.6 µm | HgCdTe (MCT) | VML10T0 VML10T4 d |
- | - | - | PDAVJ10 |
2.7 - 5.0 µm | HgCdTe (MCT) | VL5T0 | - | - | - | PDAVJ5 |
2.7 - 5.3 µm | InAsSb | - | - | - | - | PDA07P2 |

Click Image for Details |
![]() |
![]() |
![]() |
![]() |
![]() |
![]() |
![]() |
![]() |
Item # | FDS010 | FD11A | FDS10X10 | FDS100 | FDS1010 | FDS015 | FDS02 | FDS025 |
Key Feature | High Speed, UV Grade Fused Silica Window to Provide Sensitivity Down to 200 nm | Lowest Dark Current in TO-18 Can with a Window | Low Dark Current in 10 mm x 10 mm Ceramic Package | High Speed, Largest Sensor in a TO-5 Can | High Speed, Large Active Area and Mounted on an Insulating Ceramic Substrate | Highest Speed and Lowest Capacitance in a TO-46 Can with an AR-Coated Window | High Speed and Low Capacitance in a Direct Fiber-Coupled FC/PC Package | High Speed and Low Capacitance in a TO-46 Can with a Ball Lens |
Info | ![]() |
![]() |
![]() |
![]() |
![]() |
![]() |
![]() |
![]() |
Wavelength Range | 200 - 1100 nma | 320 - 1100 nm | 340 - 1100 nm | 350 - 1100 nm | 350 - 1100 nm | 400 - 1100 nm | 400 - 1100 nm | 400 - 1100 nm |
Active Area | 0.8 mm2 (Ø1.0 mm) |
1.21 mm2 (1.1 mm x 1.1 mm) |
100 mm2 (10 mm x 10 mm) |
13 mm2 (3.6 mm x 3.6 mm) |
100 mm2 (10 mm x 10 mm) |
0.018 mm2 (Ø150 µm) |
0.049 mm2 (Ø0.25 mm) |
0.049 mm2 (Ø0.25 mm) |
Rise/Fall Timeb | 1 ns / 1 ns @ 830 nm, 10 V |
400 nscc,d @ 650 nm, 0 V |
150 ns / 150 nsd @ 5 V |
10 ns / 10 nsd @ 632 nm, 20 V |
65 ns / 65 nsd @ 632 nm, 5 V |
35 ps / 200 ps @ 850 nm, 5 V |
47 ps / 246 ps @ 850 nm, 5 V |
47 ps / 246 ps @ 850 nm, 5 V |
NEP (W/Hz1/2) | 5.0 x 10-14 @ 830 nm, 10 V |
6.8 x 10-16 @ 960 nm, 0 V |
1.50 x 10-14 @ 960 nm |
1.2 x 10-14 @ 900 nm, 20 V |
2.07 x 10-13 @ 970 nm, 5 V |
8.60 x 10-15 @ 850 nm, 5 V |
9.29 x 10-15 @ 850 nm, 5 V |
9.29 x 10-15 @ 850 nm, 5 V |
Dark Current | 0.3 nA (Typ.) @ 10 V |
2.0 pA (Max) @ 10 mV |
200 pA @ 5 V | 1.0 nA (Typ.) @ 20 V |
600 nA (Max) @ 5 V |
0.03 nA (Typ.) @ 5 V |
35 pA (Typ.) @ 5 V |
35 pA (Typ.) @ 5 V |
Junction Capacitance |
6 pF (Typ.) @ 10 V | 140 pF (Typ.) @ 0 V |
380 pF @ 5 V | 24 pF (Typ.) @ 20 V |
375 pF (Typ.) @ 5 V |
0.65 pF (Typ.) @ 5 V |
0.94 pF (Typ.) @ 5 V |
0.94 pF (Typ.) @ 5 V |
Package | TO-5 | TO-18 | Ceramic | TO-5 | Ceramic | TO-46 | TO-46, FC/PC Bulkhead | TO-46 |
Compatible Sockets |
STO5S STO5P |
STO46S STO46P |
Not Available | STO5S STO5P |
Not Available | STO46S STO46P |
STO46S STO46P |
STO46S STO46P |

Click Image for Details |
![]() |
![]() |
![]() |
![]() |
![]() |
![]() |
![]() |
![]() |
Item # | FGA01 | FGA01FC | FGA015 | FDGA05 | FGA21 | FGA10 | FD05D | FD10D |
Key Feature | High Speed and Low Capacitance in a TO-46 Can with a Ball Lens | High Speed and Low Capacitance in a Direct Fiber-Coupled FC/PC Package | High Speed and Low Capacitance | High Speed, High Responsivity, and Low Capacitance | Large Active Area and High Speed | High Speed and Low Dark Current | Long Wavelength Range | Long Wavelength Range and Large Active Area |
Info | ![]() |
![]() |
![]() |
![]() |
![]() |
![]() |
![]() |
![]() |
Wavelength Range | 800 - 1700 nm | 800 - 1700 nm | 800 - 1700 nm | 800 - 1700 nm | 800 - 1700 nm | 900 - 1700 nm | 900 - 2600 nm | 900 - 2600 nm |
Active Area | 0.01 mm2 (Ø120 µm) |
0.01 mm2 (Ø120 µm) |
0.018 mm2 (Ø150 µm) |
0.196 mm2 (Ø0.5 mm) |
3.1 mm2 (Ø2 mm) | 0.79 mm2 (Ø1 mm) | 0.20 mm2 (Ø0.5 mm) |
0.79 mm2 (Ø1.0 mm) |
Rise/Fall Timea | 300 ps / 300 ps @ 5 V |
300 ps / 300 ps @ 5 V |
300 ps / 300 ps @ 1550 nm, 5 V |
2.5 ns / 2.5 ns @ 5 V |
25 ns / 25 ns @ 3 V |
10 ns / 10 ns @ 5 V |
17 ns / 17 ns @ 0 V |
25 ns / 25 ns @ 0 V |
NEP (W/Hz1/2) |
4.5 x 10-15 @ 1500 nm |
4.5 x 10-15 @ 1500 nm |
1.3 x 10-14 @ 1550 nm |
2.0 x 10-14 @ 1550 nm |
6.0 x 10-14 @ 1550 nm |
2.5 x 10-14 @ 1550 nm, 5 V |
5.0 x 10-13 @ 2300 nm |
1.0 x 10-12 @ 2300 nm |
Dark Current | 0.05 nA (Typ.) @ 5 V |
0.05 nA (Typ.) @ 5 V |
0.5 nA (Typ.) @ 5 V |
6 nA (Typ.) @ 5 V |
50 nA (Typ.) @ 1 V |
1.1 nA (Typ.) @ 5 V |
1 µA (Typ.) @ 0.5 V |
3 µA (Typ.) @ 0.5 V |
Junction Capacitance |
2.0 pF (Typ.) @ 5 V | 2.0 pF (Typ.) @ 5 V | 1.5 pF (Typ.) @ 5 V | 10 pF (Typ.) @ 5 V | 100 pF (Typ.) @ 3 V | 80 pF (Typ.) @ 5 V | 140 pF (Typ.) @ 0 V | 500 pF (Typ.) @ 0 V |
Package | TO-46 | TO-46, FC/PC Bulkhead | TO-18 | TO-46 | TO-5 | TO-5 | TO-18 | TO-18 |
Compatible Sockets |
STO46S STO46P |
STO46S STO46P |
STO46S STO46P |
STO46S STO46P |
STO5S STO5P |
STO5S STO5P |
STO46S STO46P |
STO46S STO46P |

- Dual Detector Chip Design - Si Over InGaAs - Provides Wide Detector Range
- 4-Pin TO-5 Package
- Large Active Area

Click Image for Details |
![]() |
![]() |
![]() |
![]() |
Item # | FDG03 | FDG05a | FDG50 | FDG10X10 |
Key Feature | Large Active Area in a TO-5 Can | High Speed on a Ceramic Substrate | Large Active Area in a TO-8 Can | Largest Active Area |
Info | ![]() |
![]() |
![]() |
![]() |
Wavelength Range | 800 - 1800 nm | 800 - 1800 nm | 800 - 1800 nm | 800 - 1800 nm |
Active Area | 7.1 mm2 (Ø3 mm) | 19.6 mm2 (Ø5 mm) | 19.6 mm2 (Ø5 mm) | 100 mm2 (10 mm x 10 mm) |
Rise/Fall Timeb | 600 ns / 600 ns @ 3 V | 220 ns / 220 ns @ 3 V | 220 ns / 220 ns (Typ.) @ 10 V | 10 μs (Typ.) @ 1 V |
NEP | 2.6 x 10-12 W/Hz1/2 @ 1550 nm | 4.0 x 10-12 W/Hz1/2 @ 1550 nm | 4.0 x 10-12 W/Hz1/2 @ 1550 nm | 4.0 x 10-12 W/Hz1/2 @ 1550 nmc |
Dark Current | 4.0 µA (Max) @ 1 V | 40 µA (Max) @ 3 V | 60 µA (Max) @ 5 V | 50 µA (Max) @ 0.3 V |
Junction Capacitance | 6 nF (Typ.) @ 1 V 4.5 nF (Typ.) @ 3 V |
3000 pF (Typ.) @ 3 V | 1800 pF (Max) @ 5 V 16000 pF (Max) @ 0 V |
80 nF (Typ.) @ 1 V 135 nF (Typ.) @ 0 V |
Shunt Resistance | 25 kΩ (Min) | - | 4 kΩ (Typ.) | 2 kΩ (Min) |
Package | TO-5 | Ceramic | TO-8 | Ceramic |
Compatible Sockets |
STO5S STO5P |
Not Available | STO8S STO8P |
Not Available |